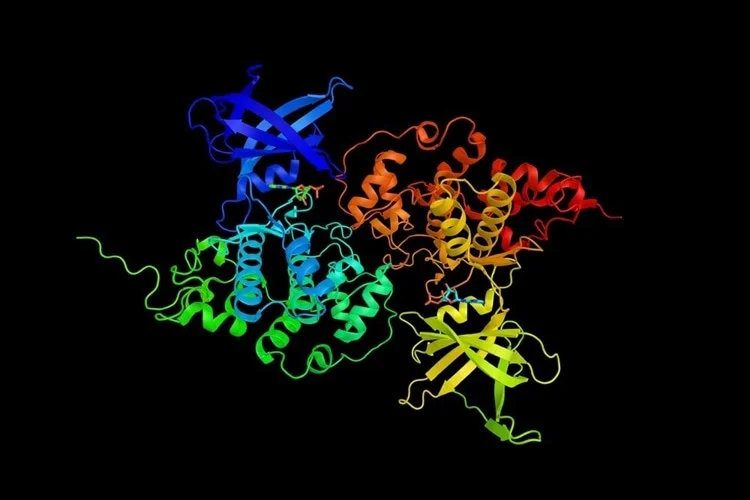
Advanced Topics in Enzymology for Bioinformatics
March 31, 2024This course provides a comprehensive understanding of enzymes and their roles in biological systems, with a focus on applications in bioinformatics. Students will gain practical knowledge of enzyme kinetics, regulation, and engineering, along with exposure to recent advancements in enzymology and its integration into bioinformatics research. The course emphasizes the use of computational tools and methods for studying enzymes, making it suitable for students interested in bioinformatics and related fields.
Introduction to Enzymes
Chemical nature of enzymes
Enzymes are biological molecules, typically proteins, that act as catalysts to facilitate and speed up biochemical reactions in living organisms. They are crucial for various metabolic processes, including digestion, energy production, and cell signaling.
The chemical nature of enzymes is characterized by several key features:
- Protein Structure: Most enzymes are proteins, although some RNA molecules can also exhibit catalytic activity (known as ribozymes). Proteins are composed of amino acids linked together in a specific sequence, which determines the protein’s three-dimensional structure and its function as an enzyme.
- Active Site: Enzymes have a region called the active site, which is typically a small crevice or pocket where the substrate (the molecule upon which the enzyme acts) binds. The active site has a specific shape that complements the shape of the substrate, allowing for a precise and selective interaction.
- Specificity: Enzymes are highly specific, meaning they usually catalyze only one type of reaction or a group of closely related reactions. This specificity is due to the precise arrangement of amino acids in the active site that enables interactions with specific substrates.
- Catalytic Mechanism: Enzymes facilitate reactions by lowering the activation energy required for the reaction to occur. They do this by stabilizing the transition state of the reaction, making it easier for the substrate molecules to reach this state and proceed to form the product.
- Regulation: Enzyme activity is often regulated to maintain optimal conditions for cellular processes. Regulation can occur through factors such as allosteric regulation, covalent modification, or changes in gene expression.
Overall, enzymes play a critical role in biochemical processes by accelerating reactions, allowing them to occur at rates necessary for life.
Naming and classification of enzymes
Enzymes are named and classified based on several criteria, including the type of reaction they catalyze, the substrate(s) they act upon, and their structure. The International Union of Biochemistry and Molecular Biology (IUBMB) has established a systematic nomenclature for enzymes, known as the Enzyme Commission (EC) numbers, which are widely used for enzyme classification. Here’s an overview:
- EC Number: The EC number is a numerical classification system that categorizes enzymes based on the type of reaction they catalyze. The number consists of four components:
- The first digit represents the class of the enzyme based on the type of reaction.
- The second digit represents the subclass of the enzyme within the class.
- The third digit represents the sub-subclass of the enzyme.
- The fourth digit is a serial number assigned to each enzyme within the sub-subclass.
For example, the EC number for the enzyme hexokinase, which catalyzes the phosphorylation of glucose to glucose-6-phosphate, is EC 2.7.1.1.
- Naming: Enzymes are often named based on the substrate(s) they act upon, followed by the type of reaction they catalyze, ending with the suffix “-ase.” For example, lactase catalyzes the hydrolysis of lactose, and lipase catalyzes the hydrolysis of lipids.
- Classification: Enzymes are classified into six main classes based on the type of reaction they catalyze:
- Oxidoreductases (EC 1): Catalyze oxidation-reduction reactions.
- Transferases (EC 2): Catalyze transfer of functional groups (e.g., amino, methyl, or phosphate groups).
- Hydrolases (EC 3): Catalyze hydrolysis reactions.
- Lyases (EC 4): Catalyze removal of groups to form double bonds or addition of groups to double bonds.
- Isomerases (EC 5): Catalyze isomerization reactions.
- Ligases (EC 6): Catalyze joining of two molecules using ATP.
- Other Classification Systems: Enzymes can also be classified based on their structure, mechanism of action, or biological function. For example, enzymes with similar structures and catalytic mechanisms are grouped into families and superfamilies.
Overall, the naming and classification of enzymes provide a systematic way to categorize and study the diverse array of biological catalysts found in living organisms.
Specificity of enzyme action
The specificity of enzyme action refers to the ability of an enzyme to select and act upon a specific substrate or group of substrates. Enzymes exhibit several types of specificity:
- Substrate Specificity: Enzymes are highly specific for their substrates. This specificity is based on the complementary shapes and chemical properties of the enzyme’s active site and the substrate. The active site of an enzyme is precisely shaped to bind to a specific substrate or group of substrates, excluding other molecules that do not fit.
- Stereochemical Specificity: Enzymes can exhibit stereochemical specificity, meaning they can distinguish between stereoisomers (molecules with the same molecular formula but different spatial arrangements of atoms). For example, enzymes involved in protein synthesis can distinguish between L-amino acids and D-amino acids.
- Regioselectivity: Enzymes can be regioselective, meaning they can selectively act on a specific region of a substrate molecule. For example, some enzymes that catalyze glycosidic bond formation in carbohydrates may be regioselective for specific positions along the carbohydrate chain.
- Chemical Specificity: Enzymes can be chemically specific, meaning they catalyze a specific chemical reaction. For example, enzymes such as amylase catalyze the hydrolysis of starch into smaller carbohydrate molecules but do not catalyze the hydrolysis of proteins or fats.
- Cofactor Specificity: Some enzymes require specific cofactors or coenzymes to catalyze reactions. Cofactor specificity refers to the requirement of a particular cofactor for the enzyme to function. For example, the enzyme catalase requires the cofactor heme to catalyze the decomposition of hydrogen peroxide into water and oxygen.
- Temperature and pH Specificity: Enzymes also exhibit specificity with respect to temperature and pH. Each enzyme has an optimal temperature and pH at which it functions most efficiently. Deviations from these optimal conditions can reduce enzyme activity.
Overall, the specificity of enzyme action is crucial for maintaining the efficiency and selectivity of biochemical reactions in living organisms.
Monomeric and oligomeric enzymes
Enzymes can be classified based on their quaternary structure, which refers to the arrangement of individual subunits in a multisubunit protein. Enzymes can exist as monomeric or oligomeric forms:
- Monomeric Enzymes: Monomeric enzymes consist of a single polypeptide chain that functions as an enzyme on its own. The entire enzyme structure is contained within this single chain. Examples of monomeric enzymes include ribonuclease A and chymotrypsinogen.
- Oligomeric Enzymes: Oligomeric enzymes are composed of multiple subunits, each of which contributes to the overall enzymatic activity. Oligomeric enzymes can be further classified based on the number of subunits:
- Dimeric: Dimeric enzymes consist of two subunits. Each subunit contributes to the active site, and the subunits can be identical or different. Examples of dimeric enzymes include lactate dehydrogenase and hemoglobin.
- Trimeric: Trimeric enzymes consist of three subunits. Each subunit contributes to the overall enzymatic activity. Examples of trimeric enzymes include tryptophan synthase and glutathione reductase.
- Tetrameric: Tetrameric enzymes consist of four subunits. Each subunit contributes to the active site, and the subunits can be arranged in different ways. Examples of tetrameric enzymes include aspartate transcarbamoylase and alcohol dehydrogenase.
- Multimeric: Enzymes with more than four subunits are classified as multimeric. These enzymes can have complex quaternary structures with multiple subunits arranged in specific patterns. Examples of multimeric enzymes include DNA polymerase and RNA polymerase.
Oligomeric enzymes often exhibit cooperative behavior, where the binding of a substrate to one subunit affects the binding of substrates to other subunits within the enzyme complex. This can enhance the efficiency of enzymatic reactions and regulate enzyme activity in response to changes in substrate concentration.
Chemical nature of enzyme catalysis
Enzyme catalysis involves several key chemical principles that govern the interaction between enzymes and their substrates, leading to the acceleration of biochemical reactions. Some of the key aspects of enzyme catalysis include:
- Activation Energy: Enzymes lower the activation energy required for a chemical reaction to occur. By stabilizing the transition state of the reaction, enzymes make it easier for substrates to reach this state and form products.
- Active Site: Enzymes have a specific region called the active site where the substrate binds. The active site is complementary in shape and charge to the substrate, allowing for precise and selective binding.
- Induced Fit Model: The induced fit model describes how enzymes undergo a conformational change upon substrate binding to achieve a better fit between the enzyme and substrate. This conformational change helps to bring catalytic groups into optimal position for catalysis.
- Catalytic Groups: Enzymes often contain specific amino acid residues or cofactors that act as catalytic groups. These groups participate in the catalytic reaction by stabilizing intermediates, facilitating bond cleavage or formation, or providing acid-base catalysis.
- Coenzymes and Cofactors: Some enzymes require coenzymes or cofactors to catalyze reactions. These molecules assist enzymes by accepting or donating chemical groups during the reaction. Examples include NAD+/NADH and ATP.
- Substrate Orientation: Enzymes can orient substrates in a way that promotes the formation of the transition state, increasing the likelihood of a successful reaction.
- Transition State Stabilization: Enzymes stabilize the transition state of a reaction, which is the highest energy state along the reaction pathway. This stabilization lowers the activation energy required for the reaction to occur.
- pH and Temperature Effects: Enzyme activity is often influenced by pH and temperature. Each enzyme has an optimal pH and temperature at which it functions most efficiently.
- Enzyme Regulation: Enzyme activity can be regulated through mechanisms such as allosteric regulation, covalent modification, and changes in gene expression. These regulatory mechanisms allow cells to control the rate of biochemical reactions based on their needs.
Overall, enzyme catalysis is a highly regulated and specific process that plays a critical role in biological processes by allowing cells to perform essential biochemical reactions with high efficiency and specificity.
Enzyme Kinetics
Derivation of Michaelis-Menten equation for single substrate enzymes
The Michaelis-Menten equation describes the rate of enzymatic reactions involving a single substrate. It is derived from the basic principles of enzyme kinetics and the assumptions of the Michaelis-Menten model. Here’s a simplified derivation:
- Assumptions:
- The enzyme-substrate (ES) complex formation is reversible.
- The rate of ES complex formation is much faster than its breakdown (k1 ≫ k-1).
- The ES complex is converted to product (E + S → P + E) at a rate determined by k2.
Concepts of Km and Vmax
Enzyme turnover number (Kcat)
The enzyme turnover number, often denoted as �cat, is a measure of the catalytic efficiency of an enzyme. It represents the number of substrate molecules converted to product per enzyme active site per unit time when the enzyme is saturated with substrate.
Kinetics of zero and first-order reactions
Zero-order and first-order reactions refer to the rate of change of a reaction with respect to the concentration of reactants. Here’s a brief overview of each:
In summary, zero-order reactions have a constant rate of reaction, while first-order reactions have a rate of reaction that is directly proportional to the concentration of the reactant. The integrated rate laws can be used to determine the concentration of reactants at different times during the reaction.
Types of inhibitors and their effects on enzyme-catalyzed reactions
Inhibitors are molecules that bind to enzymes and reduce their activity. They can be classified into several types based on their mechanism of action and effects on enzyme-catalyzed reactions. Here are the main types of inhibitors and their effects:
- Competitive Inhibitors:
- Competitive inhibitors bind to the active site of the enzyme, competing with the substrate for binding.
- They do not affect the maximum rate of the reaction (�max), but they increase the apparent �� (the concentration of substrate at which the reaction rate is half of �max).
- Competitive inhibitors can be overcome by increasing the concentration of substrate.
- Non-competitive Inhibitors:
- Non-competitive inhibitors bind to the enzyme at a site other than the active site, known as the allosteric site.
- They inhibit enzyme activity by causing a conformational change in the enzyme that reduces its catalytic activity.
- Non-competitive inhibitors do not affect the �� but reduce the �max of the enzyme.
- Uncompetitive Inhibitors:
- Uncompetitive inhibitors bind to the enzyme-substrate complex, forming a ternary complex.
- They inhibit the enzyme by preventing the release of the product, effectively reducing both the �� and the �max of the enzyme.
- Mixed Inhibitors:
- Mixed inhibitors bind to the enzyme at a site other than the active site, but they can bind to either the free enzyme or the enzyme-substrate complex.
- Mixed inhibitors can either increase or decrease the �� and �max depending on their affinity for the enzyme and the enzyme-substrate complex.
- Irreversible Inhibitors:
- Irreversible inhibitors bind covalently to the enzyme, permanently inactivating it.
- They are often used as drugs to inhibit specific enzymes in the body.
The effects of inhibitors on enzyme-catalyzed reactions depend on their mechanism of action and the concentration of both the inhibitor and the substrate. Understanding the types of inhibitors and their effects is important in drug design and understanding enzyme kinetics.
Multi-substrate enzyme-catalyzed reactions
Multi-substrate enzyme-catalyzed reactions involve enzymes that catalyze reactions involving more than one substrate. These reactions can follow different mechanisms, and the kinetics can be more complex compared to single-substrate reactions. Here are some common types of multi-substrate enzyme-catalyzed reactions and their characteristics:
- Sequential Reactions:
- In sequential reactions, all substrates must bind to the enzyme before any products are released.
- There are two main types of sequential reactions:
- Ordered sequential reactions: Substrates must bind in a specific order.
- Random sequential reactions: Substrates can bind in any order.
- Ping-Pong Reactions (Double Displacement Reactions):
- In ping-pong reactions, one or more products are released before all substrates have bound to the enzyme.
- The enzyme alternates between two different forms during the reaction, each with a different substrate or product bound.
- Bi-Bi Reactions:
- Bi-Bi reactions involve the binding of two substrates and the release of two products.
- There are two main types of Bi-Bi reactions:
- The ternary complex mechanism: Both substrates bind to the enzyme before any product is released.
- The double-displacement mechanism: One or more products are released before all substrates have bound.
- Multi-Substrate Enzyme Kinetics:
- The kinetics of multi-substrate enzyme-catalyzed reactions can be more complex than single-substrate reactions.
- The Michaelis-Menten equation can be extended to include multiple substrates, leading to more complex rate equations.
- The kinetic parameters such as �� and �max can vary depending on the concentrations of multiple substrates.
Understanding the mechanisms and kinetics of multi-substrate enzyme-catalyzed reactions is important for studying enzyme function and designing inhibitors or activators of these enzymes for therapeutic purposes.
Enzyme Regulation
General mechanisms of enzyme regulation
Enzyme regulation refers to the control of enzyme activity in cells, which is crucial for maintaining homeostasis and responding to changes in the environment. Enzyme regulation can occur at various levels, including gene expression, post-translational modifications, and allosteric regulation. Here are some general mechanisms of enzyme regulation:
- Gene Expression:
- Enzyme activity can be regulated at the level of gene expression. This includes the transcription of genes encoding enzymes and the translation of mRNA into protein.
- Factors that regulate gene expression include transcription factors, epigenetic modifications (e.g., DNA methylation, histone modifications), and environmental signals.
- Post-translational Modifications (PTMs):
- PTMs can regulate enzyme activity by altering the structure, stability, or localization of the enzyme. Common PTMs include phosphorylation, acetylation, methylation, and ubiquitination.
- For example, phosphorylation of enzymes by protein kinases can activate or deactivate enzyme activity, while dephosphorylation by protein phosphatases can reverse this effect.
- Allosteric Regulation:
- Allosteric regulation involves the binding of a regulatory molecule to a site on the enzyme (allosteric site) that is distinct from the active site. This binding can either activate or inhibit enzyme activity.
- Allosteric regulators can be positive (activators) or negative (inhibitors) and can modulate enzyme activity in response to changes in cellular conditions.
- Feedback Inhibition:
- Feedback inhibition is a type of allosteric regulation in which the end product of a metabolic pathway binds to an enzyme early in the pathway, inhibiting its activity.
- This mechanism helps to prevent the over-accumulation of metabolic intermediates and regulates the rate of the pathway in response to the levels of the end product.
- Covalent Modification:
- Covalent modification involves the addition or removal of chemical groups to enzyme molecules. This can alter enzyme activity by changing the enzyme’s conformation or its ability to bind substrates.
- Examples include acetylation, methylation, and glycosylation.
- Protein-Protein Interactions:
- Enzyme activity can be regulated by interactions with other proteins. These interactions can stabilize or destabilize the enzyme, alter its subcellular localization, or modulate its ability to interact with substrates or cofactors.
These mechanisms of enzyme regulation allow cells to fine-tune enzyme activity in response to changing conditions, ensuring that metabolic pathways are tightly regulated and coordinated.
Sigmoidal and allosteric enzymes
Sigmoidal enzymes, also known as allosteric enzymes, exhibit a sigmoidal (S-shaped) saturation curve in enzyme kinetics plots, unlike the hyperbolic curve seen in Michaelis-Menten enzymes. Allosteric enzymes have multiple subunits and contain allosteric sites, which are distinct from the active sites where substrates bind. Here are some key characteristics of allosteric enzymes:
- Cooperative Binding: Allosteric enzymes show cooperative binding of substrates, meaning that the binding of one substrate molecule affects the binding of subsequent substrate molecules. This results in the sigmoidal curve in the enzyme kinetics plot.
- Allosteric Sites: Allosteric enzymes have allosteric sites where regulatory molecules, called allosteric effectors, can bind. Allosteric effectors can be either activators or inhibitors, and their binding alters the enzyme’s affinity for substrates and/or its catalytic activity.
- Conformational Changes: Binding of allosteric effectors to the allosteric sites induces conformational changes in the enzyme structure. These changes can affect the enzyme’s active site, making it more or less accessible to substrates.
- Regulation of Metabolic Pathways: Allosteric enzymes play a crucial role in regulating metabolic pathways. By responding to changes in the concentrations of substrates or products in a pathway, allosteric enzymes help maintain metabolic homeostasis.
- Feedback Inhibition: Some allosteric enzymes are subject to feedback inhibition, where the end product of a metabolic pathway acts as an allosteric inhibitor of an enzyme early in the pathway. This helps regulate the rate of the pathway based on the concentration of the end product.
- Examples: Examples of allosteric enzymes include hemoglobin (which exhibits cooperative binding of oxygen), aspartate transcarbamoylase (which is involved in pyrimidine biosynthesis), and phosphofructokinase-1 (which regulates glycolysis).
The sigmoidal kinetics of allosteric enzymes allow for fine-tuned regulation of metabolic pathways, ensuring that they respond appropriately to changing cellular conditions and metabolic demands.
Hill and Scatchard plots
Hill and Scatchard plots are graphical representations used in the analysis of cooperative binding of ligands to proteins, such as enzymes or receptors. These plots are particularly useful for studying allosteric enzymes or proteins with multiple binding sites. Here’s a brief overview of each:
- Hill and Scatchard plots are graphical representations used in the analysis of cooperative binding of ligands to proteins, such as enzymes or receptors. These plots are particularly useful for studying allosteric enzymes or proteins with multiple binding sites. Here’s a brief overview of each:
- Hill Plot:
- The Hill plot is used to determine the cooperativity of binding in a system. It plots the logarithm of the fractional saturation of a protein (Y-axis) against the logarithm of the ligand concentration (X-axis).
- The slope of the Hill plot is related to the Hill coefficient (�), which is a measure of cooperativity. A slope greater than 1 indicates positive cooperativity, while a slope less than 1 indicates negative cooperativity.
- Hill Plot:
Hill and Scatchard plots are valuable tools for characterizing the binding properties of proteins and understanding the mechanisms of cooperative binding. They provide insights into the kinetics and thermodynamics of ligand binding, particularly in systems with complex binding behavior.
Positive and negative cooperativity with examples (e.g., aspartate transcarbamoylase & phosphofructokinase)
Positive Cooperativity:
- Positive cooperativity occurs when the binding of one ligand to a multisubunit protein increases the affinity of the other subunits for the ligand.
- An example of positive cooperativity is seen in hemoglobin, a tetrameric protein composed of two α and two β subunits. When one oxygen molecule binds to one of the four heme groups, it increases the affinity of the remaining heme groups for oxygen, making it easier for the other subunits to bind oxygen.
Negative Cooperativity:
- Negative cooperativity occurs when the binding of one ligand to a multisubunit protein decreases the affinity of the other subunits for the ligand.
- An example of negative cooperativity is seen in the enzyme aspartate transcarbamoylase (ATCase), which catalyzes the committed step in the biosynthesis of pyrimidines. ATCase is a homotropic allosteric enzyme, meaning its substrate, carbamoyl phosphate, acts as an allosteric regulator. The binding of carbamoyl phosphate to one subunit decreases the affinity of the other subunits for the substrate, leading to negative cooperativity.
Phosphofructokinase (PFK):
- PFK is an enzyme involved in glycolysis, catalyzing the conversion of fructose-6-phosphate to fructose-1,6-bisphosphate.
- PFK exhibits positive cooperativity with its substrate ATP. As ATP concentration increases, the affinity of PFK for ATP increases, allowing PFK to become more active when ATP levels are high, which is indicative of a high-energy state in the cell.
In summary, positive cooperativity enhances the binding of additional ligands to a multisubunit protein, while negative cooperativity decreases the binding affinity. These mechanisms allow enzymes and other proteins to respond to changing cellular conditions and regulate their activity accordingly.
Applied Enzymology
Applications of enzymology in bioinformatics
Enzymology plays a significant role in bioinformatics, particularly in the analysis of biological sequences, protein structure, and metabolic pathways. Some key applications of enzymology in bioinformatics include:
- Sequence Analysis: Enzymes are often used in bioinformatics tools for sequence alignment, motif searching, and phylogenetic analysis. Enzymes such as DNA polymerases and restriction enzymes are essential for techniques like PCR and DNA sequencing.
- Protein Structure Prediction: Enzymes are used in bioinformatics tools for predicting protein structures based on sequence information. Understanding enzyme structures is crucial for predicting their function and designing drugs that target specific enzymes.
- Metabolic Pathway Analysis: Enzymes are central to metabolic pathways, and their activities can be analyzed using bioinformatics tools to understand the flow of metabolites and the regulation of metabolism. This information is valuable for drug discovery and metabolic engineering.
- Enzyme Function Prediction: Bioinformatics tools can predict the function of uncharacterized enzymes based on their sequence and structural features. This helps in identifying enzymes with specific activities for various applications.
- Drug Design: Enzymes are common targets for drug design, and bioinformatics tools are used to analyze enzyme structures, predict binding sites, and design inhibitors or activators that modulate enzyme activity.
- Biological Database Analysis: Enzyme data is stored in various biological databases, and bioinformatics tools are used to analyze and annotate enzyme sequences, structures, and functions in these databases.
- Evolutionary Studies: Enzyme sequences are used in evolutionary studies to understand the relationships between different species and the evolution of enzyme function over time.
Overall, enzymology is an integral part of bioinformatics, providing essential tools and insights for understanding biological processes, predicting protein function, and designing novel therapeutics.
Structure and function of enzymes
Enzymes are biological catalysts that accelerate chemical reactions in living organisms. They are typically proteins, although some RNA molecules can also exhibit catalytic activity (known as ribozymes). Enzymes have a complex three-dimensional structure that is essential for their function. Here’s a brief overview of the structure and function of enzymes:
- Structure of Enzymes:
- Enzymes are made up of long chains of amino acids that fold into unique three-dimensional shapes.
- The structure of an enzyme is critical to its function, as the active site, where the substrate binds and the catalytic reaction occurs, is a specific region of the enzyme’s structure.
- Enzymes can have additional structural features, such as allosteric sites (sites that bind regulatory molecules) and cofactor-binding sites (sites that bind non-protein molecules necessary for catalytic activity).
- Function of Enzymes:
- Enzymes catalyze chemical reactions by lowering the activation energy required for the reaction to occur. They do this by stabilizing the transition state of the reaction, making it easier for the reaction to proceed.
- Enzymes are highly specific, meaning that they catalyze specific reactions with specific substrates. This specificity is due to the precise arrangement of amino acids in the active site that allows it to bind only to certain molecules.
- Enzymes can be regulated in various ways, such as through allosteric regulation (binding of regulatory molecules to allosteric sites), covalent modification (addition or removal of chemical groups), and proteolytic cleavage (cleavage of the enzyme into smaller fragments).
- Classification of Enzymes:
- Enzymes are classified into several classes based on the type of reaction they catalyze. The six main classes of enzymes are oxidoreductases, transferases, hydrolases, lyases, isomerases, and ligases.
- Each enzyme class is further subdivided into families and subfamilies based on sequence and structural similarities.
In summary, enzymes are essential biological molecules that play a crucial role in catalyzing a wide range of biochemical reactions. Their structure allows them to catalyze specific reactions with high efficiency and specificity, making them key players in the maintenance of life processes.
Enzyme engineering with examples
Enzyme engineering, also known as protein engineering, involves modifying enzymes to improve their properties or create new functions. This field has applications in various industries, including pharmaceuticals, biofuels, and food production. Here are some examples of enzyme engineering:
- Directed Evolution: Directed evolution is a powerful technique used to engineer enzymes with improved properties. It involves creating a library of enzyme variants with random mutations and selecting those variants with desired traits through screening or selection processes. One example is the engineering of the enzyme lipase for improved activity in non-aqueous solvents, which is useful in biodiesel production.
- Rational Design: Rational design involves making specific changes to an enzyme based on an understanding of its structure and function. For example, researchers have engineered enzymes involved in cellulose degradation to improve their efficiency in biofuel production from plant biomass.
- Immobilization: Enzyme immobilization involves attaching enzymes to a solid support, which can improve enzyme stability, reusability, and ease of separation from the reaction mixture. Immobilized enzymes are used in various industrial processes, such as the production of pharmaceuticals and food products.
- Protein Fusion: Protein fusion involves combining an enzyme with another protein or peptide to improve its stability, solubility, or activity. For example, fusion of enzymes with a cellulose-binding domain can improve their binding to cellulose substrates, enhancing their efficiency in biomass conversion.
- Metabolic Engineering: Metabolic engineering involves modifying metabolic pathways in microorganisms to improve the production of desired compounds. Enzyme engineering plays a crucial role in metabolic engineering by optimizing enzyme activities and substrate specificities. An example is the engineering of enzymes involved in the biosynthesis of amino acids or vitamins in bacteria for increased production yields.
- Designer Enzymes: Designer enzymes are engineered enzymes with novel functions not found in nature. For example, researchers have engineered enzymes capable of catalyzing reactions that do not occur in nature, such as the synthesis of unnatural amino acids or pharmaceutical compounds.
Enzyme engineering continues to be a rapidly evolving field with a wide range of applications in biotechnology, medicine, and industry. The ability to tailor enzymes for specific purposes holds great promise for addressing global challenges in health, energy, and sustainability.
Enzymeomics
Genomic and proteomic approaches have revolutionized the study of enzymes by providing comprehensive insights into their identification, characterization, and evolution. Here’s how these approaches are used:
- Enzyme Identification:
- Genomic approaches involve sequencing the entire genome of an organism to identify genes encoding enzymes. Bioinformatics tools are then used to predict the functions of these enzymes based on sequence homology to known enzymes.
- Proteomic approaches involve the large-scale identification of proteins, including enzymes, expressed in a cell, tissue, or organism. Techniques such as mass spectrometry are used to identify and quantify proteins in complex mixtures.
- Enzyme Characterization:
- Genomic and proteomic approaches are used to characterize the biochemical properties of enzymes, including substrate specificity, catalytic activity, and regulation.
- Proteomics can also provide information about the post-translational modifications of enzymes, which can affect their activity and function.
- Enzyme Evolution:
- Genomic approaches are used to study the evolution of enzymes by comparing the sequences of homologous enzymes from different species. This information can help trace the evolutionary history of enzymes and predict their functions in different organisms.
- Proteomic approaches can also provide insights into enzyme evolution by comparing the expression patterns of enzymes in different species or under different conditions.
- Systems Biology:
- Genomic and proteomic approaches are used in systems biology to study enzymes in the context of larger biological networks. This approach allows researchers to understand how enzymes interact with other molecules in the cell and how their activities are regulated.
Overall, genomic and proteomic approaches have greatly expanded our understanding of enzymes, providing a wealth of information that can be used to engineer enzymes for various biotechnological applications and to develop new therapeutic strategies.
Computational Enzymology
Computational enzymology involves the use of computational methods, such as molecular modeling and simulation, to study enzyme structure, function, and dynamics. These methods complement experimental techniques and provide detailed insights into enzyme behavior at the molecular level. Here are some key aspects of computational enzymology:
- Enzyme Structure Prediction: Computational methods can be used to predict the three-dimensional structure of enzymes based on their amino acid sequences. This is particularly useful for enzymes whose structures have not been experimentally determined.
- Enzyme-Substrate Interactions: Molecular docking simulations can be used to study the interactions between enzymes and their substrates. This can help identify key amino acid residues involved in substrate binding and catalysis.
- Enzyme Mechanism: Computational methods, such as quantum mechanics/molecular mechanics (QM/MM) simulations, can be used to study the detailed mechanism of enzyme-catalyzed reactions. These simulations can provide insights into the transition states and reaction intermediates involved in catalysis.
- Enzyme Dynamics: Molecular dynamics simulations can be used to study the dynamics of enzymes, including conformational changes that occur during catalysis. These simulations can reveal important insights into enzyme function and regulation.
- Drug Design: Computational methods are used in drug design to identify potential enzyme inhibitors or activators. Virtual screening techniques can be used to screen large libraries of compounds to identify molecules that bind to the enzyme active site with high affinity.
- Enzyme Engineering: Computational methods can be used in enzyme engineering to design enzymes with improved properties. This can involve rational design, where specific amino acid changes are made based on structural insights, or directed evolution, where enzyme variants are generated and screened computationally.
Overall, computational enzymology is a powerful tool for studying enzymes and has applications in drug discovery, biotechnology, and understanding enzyme function in health and disease.
Metabolic Pathway Analysis
Metabolic pathway analysis is a critical application of enzymology that involves studying and modeling the biochemical reactions that occur in living organisms. Enzymes play a central role in metabolic pathways by catalyzing these reactions. Here are some key applications of enzymology in analyzing and modeling metabolic pathways:
- Enzyme Identification: Enzymes involved in metabolic pathways can be identified using genomic and proteomic approaches. This information is crucial for understanding how different enzymes contribute to the overall function of a pathway.
- Pathway Reconstruction: Enzymes and their corresponding reactions can be used to reconstruct metabolic pathways. This involves mapping the flow of metabolites through a series of enzymatic reactions, providing a holistic view of cellular metabolism.
- Flux Balance Analysis (FBA): FBA is a computational approach used to model and analyze metabolic pathways. It calculates the flow of metabolites through a network of enzymatic reactions to predict cellular phenotypes, such as growth rate or metabolite production, under different conditions. Enzyme kinetics and stoichiometry are key parameters used in FBA models.
- Pathway Enrichment Analysis: Pathway enrichment analysis is used to identify metabolic pathways that are significantly enriched with differentially expressed enzymes under specific conditions. This helps in understanding how metabolic pathways are regulated in response to environmental changes or disease states.
- Metabolic Engineering: Enzymology is used in metabolic engineering to manipulate metabolic pathways for the production of valuable compounds, such as biofuels, pharmaceuticals, and chemicals. This involves modifying enzyme activities or introducing new enzymes to redirect metabolic flux towards desired products.
- Drug Target Identification: Enzymes involved in metabolic pathways are often targeted for drug development. Enzymology is used to identify enzymes that are essential for pathogen survival or involved in disease pathways, providing potential targets for drug intervention.
In summary, enzymology plays a crucial role in the analysis and modeling of metabolic pathways, providing insights into how enzymes function within these pathways and how they can be manipulated for various biotechnological and therapeutic applications.
Systems Biology
Systems biology is an interdisciplinary field that aims to understand complex biological systems by integrating experimental and computational approaches. Enzymology plays a crucial role in systems biology by providing detailed insights into the catalytic activities and regulatory mechanisms of enzymes within biological systems. Here’s how enzymatic data is integrated into systems biology approaches:
- Enzyme Kinetics: Enzyme kinetics data, such as Michaelis-Menten parameters (��, �max, �cat), are used to model the behavior of enzymes within metabolic pathways. This information helps in understanding how enzymes regulate the flux of metabolites through a pathway and how they respond to changes in substrate concentration or enzyme activity.
- Metabolic Pathway Analysis: Enzyme-catalyzed reactions are the building blocks of metabolic pathways. By integrating enzymatic data into metabolic network models, systems biologists can simulate the flow of metabolites through pathways and predict how changes in enzyme activity or expression affect cellular metabolism.
- Enzyme Regulation: Enzyme regulation is a key aspect of cellular homeostasis. Enzymatic data is used to model the regulatory mechanisms that control enzyme activity, such as allosteric regulation, post-translational modifications, and enzyme-substrate interactions. This information helps in understanding how cells respond to internal and external signals.
- Enzyme-Protein Interactions: Enzymes often interact with other proteins to form complexes that regulate their activity or localization. Enzymatic data is integrated with protein-protein interaction networks to model the dynamics of these complexes and their role in cellular processes.
- Disease Mechanisms: Enzymes are often implicated in disease mechanisms, either as causative agents or as targets for therapy. Enzymatic data is used in systems biology to model how changes in enzyme activity or expression contribute to disease progression and to identify potential drug targets.
Overall, enzymatic data is a valuable resource in systems biology, providing insights into the molecular mechanisms that underlie complex biological systems. Integrating enzymatic data with other omics data (such as genomics, transcriptomics, and proteomics) allows for a more comprehensive understanding of how biological systems function and how they can be manipulated for various applications.
Synthetic Biology
Synthetic biology is a field that focuses on the design and construction of new biological parts, devices, and systems, as well as the redesign of existing biological systems for useful purposes. Enzymes play a crucial role in synthetic biology, as they can be engineered for novel functions and applications in bioinformatics and biotechnology. Here’s how enzymes are engineered in synthetic biology:
- Directed Evolution: Directed evolution is a powerful technique used to engineer enzymes with novel or improved functions. It involves generating a library of enzyme variants with random mutations and selecting those variants with desired traits through screening or selection processes. Directed evolution has been used to engineer enzymes for various applications, such as improved catalytic activity, substrate specificity, or stability.
- Rational Design: Rational design involves making specific changes to an enzyme based on an understanding of its structure and function. This can include site-directed mutagenesis to introduce specific amino acid changes that enhance enzyme activity or substrate specificity. Rational design is often guided by computational modeling and bioinformatics tools.
- Metabolic Engineering: Metabolic engineering involves modifying metabolic pathways in microorganisms to produce valuable compounds. Enzymes play a key role in metabolic engineering, as they can be engineered to enhance the production of desired products or to introduce new pathways for the synthesis of novel compounds.
- Biocatalysis: Enzymes are used as biocatalysts in various industrial processes, such as the production of pharmaceuticals, chemicals, and biofuels. Enzymes can be engineered to improve their catalytic efficiency, substrate specificity, or stability, making them more suitable for industrial applications.
- Enzyme-Substrate Engineering: Enzymes can be engineered to recognize and bind to new substrates that they would not naturally interact with. This can be useful for designing enzymes that catalyze specific chemical reactions for bioinformatics and biotechnology applications.
Overall, enzyme engineering in synthetic biology enables the design of enzymes with tailored functions and properties for a wide range of applications, from bioinformatics to biotechnology, paving the way for new advancements in medicine, agriculture, and industry.
Drug Discovery
Enzymes play a crucial role in drug discovery and development, both as targets for therapeutic intervention and as tools for drug screening and development. Here’s how enzymes are involved in drug discovery:
- Enzymes as Drug Targets: Many diseases are caused by dysregulation of enzyme activity. Enzymes involved in disease pathways can be targeted with drugs to modulate their activity and treat the disease. For example, inhibitors of enzymes involved in cancer cell proliferation or viral replication are used as anticancer or antiviral drugs.
- Enzyme Inhibitors: Enzyme inhibitors are molecules that bind to enzymes and inhibit their activity. These inhibitors can be used as drugs to target specific enzymes involved in disease processes. There are several types of enzyme inhibitors, including competitive inhibitors, non-competitive inhibitors, and allosteric inhibitors.
- Enzyme-Based Assays: Enzymes are used in high-throughput screening assays to identify potential drug candidates. These assays involve measuring the activity of an enzyme in the presence of different compounds to identify molecules that can modulate enzyme activity. Enzyme-based assays are widely used in drug discovery to screen large libraries of compounds for potential drug candidates.
- Enzyme Engineering: Enzyme engineering is used to modify enzymes for specific drug discovery applications. For example, enzymes can be engineered to increase their stability, substrate specificity, or catalytic efficiency, making them more suitable for use in drug screening assays.
- Protease Inhibitors: Proteases are enzymes that catalyze the cleavage of proteins. Protease inhibitors are used as drugs to inhibit the activity of specific proteases involved in diseases such as HIV/AIDS and hepatitis C.
- Enzyme Kinetics: Enzyme kinetics is used in drug discovery to study the interactions between enzymes and inhibitors. By measuring the kinetics of enzyme inhibition, researchers can determine the potency and mechanism of action of enzyme inhibitors, which is important for drug development.
Overall, enzymes play a critical role in drug discovery and development, both as targets for therapeutic intervention and as tools for screening and developing new drugs. Understanding the role of enzymes in disease processes and developing drugs that target specific enzymes is key to developing effective treatments for a wide range of diseases.